. Introduction
Landslide displacements can occur with a very broad range of velocities, from catastrophic movements (e.g.Baroň et al., 2011 : in 2010, in the Czech Carpathians colluvia were transported 253 m downslope during only a few hours — Fig. 1a) compared with slow-moving landslides, where the rate of movement may be less than a centimetre per year (Noferini et al., 2007; Massey et al., 2013). In the long-term, however, even such a subtle mass transfer may become significant for both the evolution of hillslopes and hazard and risk assessment. Slow displacements can be deep-seated or recorded in the near surface layer (Peyret et al., 2008; Macfarlane, 2009; Kaunda, 2010; Di Maio et al., 2013; De Vita et al., 2013; García-Davalillo et al., 2014; Grana and Tommasi, 2014), and may characterize various kinematic mechanisms, from rotational sliding through simple translation to flowslides (Malet et al., 2004; Greif et al., 2006; Ranalli et al., 2010; Herrera et al., 2013; Massey et al., 2013). Likewise, such displacements have been recorded in a variety of materials, including regolith mantles (Grana and Tommasi, 2014), flysch rocks, marls, limestones and mudstones (De Vita et al., 2013; Grana and Tommasi, 2014), travertines and clays (Wan and Kwong, 2002;Malet et al., 2004; Vlcko, 2004; Ranalli et al., 2010; Comegna et al., 2013; Di Maio et al., 2013) and schists (Macfarlane, 2009; García-Davalillo et al., 2014). Slow movement of colluvium may signify an early stage in landslide development, i.e. occur prior to the main phase of displacement (Peyret et al., 2008), or follow the main phase as a secondary relaxation movement (Macfarlane, 2009;Fernández-Merodo et al., 2014 ; Massey et al., 2013).
Fig. 1
a) Trees tilted and fallen during a single, abrupt landslide event on Girova Mt in 2010 (Western Carpathians, Czech Republic), b) Trees tilted upslope by slow movement of the Suchawa landslide and showing the pattern of upslope eccentricity developed in a spruce tree (Picea abies Karst.) tilted upslope.
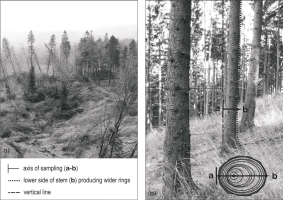
Various methods have been tried to monitor slow movements of landslide bodies. These include satellite monitoring (Peyret et al., 2008; Cascini et al., 2009; Massey et al., 2013; García-Davalillo et al., 2014), remote sensing (Hervás et al., 2003; Noferini et al., 2007; Razak et al., 2011; De Vita et al., 2013), repetitive geodetic surveys (Peyret et al., 2008; Macfarlane, 2009; De Vita et al., 2013), and hydrogeological monitoring (Greif et al., 2006; Macfarlane, 2009; Klimeš et al., 2012; Di Maio et al., 2013; De Vita et al., 2013; Grana and Tommasi, 2014). Dendrochronology provides another method, with the advantage that this usually covers a longer time period than any of the instrumental methods. In this approach, changes in wood anatomy of trees growing on landslide-affected slopes are analyzed and interpreted in terms of external disturbance induced by slope instability (e.g. Shroder, 1980; Lang et al., 1999; Braam et al., 1987; Corominas and Moya, 1999,2010 ; Fantucci and Sorriso-Valvo, 1999; Stefanini, 2004; Migoń et al., 2010; Pánek et al., 2011; Lopez Saez et al., 2012a and 2012b; Wistuba et al., 2013).
Trees are very sensitive indicators of landsliding. They can be tilted even during very slight ground movements (Malik and Wistuba, 2012) and, after tilting, develop eccentric annual rings which help us to date landsliding events (Braam et al., 1987; Filion et al., 1991; Fantucci and McCord, 1995; Stefanini, 2004; Corominas and Moya, 2010). In response to stress trees produce normal, reaction, opposite and eccentric wood (e.g. Yamaguchi et al., 1980; Kwon et al., 2001; Du et al., 2004). In the case of coniferous species post-tilting tree rings developed on the lower side of a stem are wider than respective rings developed on the opposite, upper side (e.g. Fig. 1b — upslope eccentricity in a tree tilted upslope).
In natural environmental conditions in Central Europe spruce stands can reach the age of 200–300 years, therefore dendrochronological reconstructions of landslide activity in the last few tens to hundreds of years are possible. Dendrochronological methods provide annual resolution of data of high accuracy due to the seasonal pattern of tree growth. Since the tree stands usually cover large areas results even for whole mountain massifs and ranges can be obtained. Eccentricity indicex of Norway spruce growth was developed to date landslide activity through the comparison with reference trees growing on neighbouring landslide-free slopes (Wistuba et al., 2013).
The aim of this study is to show how the dendrogeomorphological method can be employed to detect slow ground displacements and reveal the activity status of apparently relict landslides. The area chosen is the Kamienne Mountains, in south-west Poland, where many hillslopes show clear geomorphic signatures of landslides, but no surface displacements have been reported or described in historical times, i.e. in the last 300 years or so. This study examines possible triggering factors for slope instability, particularly those that could be linked to episodes of high rainfall. We also intend to validate the usefulness of tree-ring eccentricity studies as an approach for the detection and analysis of activity patterns of slow moving landslides.
. Study Area
Regional Geology And Landforms
The study area is located in the Kamienne (Stone) Mountains, a range in the centre of the Sudetes Mountains, Central Europe (Fig. 2a). This area is known for the widespread occurrence of landslide-affected terrain, apparently of different ages (Migoń et al., 2010, 2014; Kacprzak et al., 2013). The bedrock of the Kamienne Mts. is composed of igneous and sedimentary rocks of late Carboniferous and early Permian age (Awdankiewicz, 1999; Fig. 2b). The former comprise both extrusive and sub-volcanic bodies of rhyolite, trachyandesite, and rhyolitic tuff, which overlie or intrude sedimentary packages of sandstones, mudstones and claystones. Juxtaposition of rigid volcanic rocks and competent sedimentary rocks, along with significant local topographic relief, sets the stage for slope instability.
Fig. 2
a) Location of the study area in Central Europe and Poland, b) Location of studied landslide slopes in the Kamienne Mts.
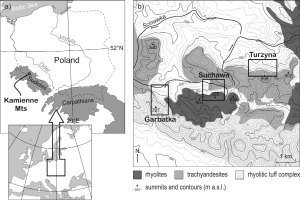
The area chosen for the detailed study embraces the east-central part of the Kamienne Mountains, which is also the highest part of the range and culminating in Mt Waligóra (936 m a.s.l.) (Fig. 2b). The terrain is considerably dissected, with V-shaped valleys separating long sinuous ridges and steep-sided conical hills. Very little planar relief is present along water divides. Mean slope angles fall within the range of 20–40°, and locally thin regolith-covered slopes are as steep as 60°. Except for footslope settings, slopes in the area carry a rather shallow regolith cover, less than 2 m thick (Migoń et al., 2010; Kacprzak et al., 2013).
The Kamienne Mountains are situated in the humid temperate zone, with a mean annual temperature around 5.5°C. Mean annual precipitation at the nearby weather station in Mieroszów operated by the Institute of Meteorology and Water Management in Wrocław (IMGW), was 776 mm in the period 1977–2007, although the amount of rainfall varied considerably from year to year. For example, values as low as 534 mm in 1982 and as high as 977 mm in 2007 were recorded. Within the 30-year period of observations, the monthly rainfall in summer (May September) exceeded 100 mm in 36 instances, occasionally rising above 250 mm. An absolute monthly maximum was recorded in July 1997 (344 mm). The station in Mieroszów (located 2 km from the Garbatka landslide, 5 and 7 km from Suchawa and Turzyna landslides) as ca. 500 m a.s.l., hence the rainfall totals on the landslide affected slopes, situated at 550–870 m a.s.l., are likely to be raised by 10–15% in the highest locations. Snow cover is present for 100–120 days in a year, a reaching maximum thickness of ca. 1 m (Migoń et al., 2010).
Natural vegetation in the Kamienne Mountains is a mixed forest, with European beech (Fagus sylvatica L.), Sycamore maple (Acer pseudoplatanus), hornbeam (Carpinus betulus) and Norway spruce (Picea abies Karst) as dominant species. However, such a forest composition has survived in a few places only, having been replaced by Norway spruce monocultures at the turn of the 20th century, after centuries of intense logging.
Detailed studies of Norway spruce response to ground instability were carried out at three locations, named here after as Suchawa, Turzyna, and Garbatka, from the name of the nearest mountain peakes. Each site exhibits different geomorphic signatures of landslide activity. In every case, Norway spruce forest stands grow on all the landslide-affected slopes.
Suchawa Landslide
Mt Suchawa (928 m a.s.l.; Fig. 2b) the second highest peak in the Kamienne Mountains, overlook the deep Sokołowiec valley to the north. Local relief attains 300 m, while terrain inclinations vary from 30–40° on the upper slope to less than 10° near the footslope, although with much local diversity. The summit and the upper slopes of Mt Suchawa are built of densely jointed rhyolites, with joint spacing usually in the order of 15–20 cm, but locally as low as 5 cm. The thickness of the rhyolite body is estimated as ca. 100–150 m (Awdankiewicz, 1999), while sedimentary clastic rocks occur at lower elevation.
The northern slopes of Mt Suchawa host one of the most extensive and complex landslides in the range extending more than 0.5 km across the slope (Fig. 3a). The whole landslide assemblage is crowned by a distinctive head scarp more than 40 m high and occupies the altitude belt 880–680 m a.s.l. A few separate types of displacement have been distinguished within the complex through landform analysis, and different ages have been proposed on the basis of evident differences in soil development and characteristics (Kacprzak et al., 2013).
Extensive slope collapse has occurred on the western side of the mountain, leaving an arcuate rock scarp that feeds an active scree slope (Remisz et al., 2009; Remisz and Bijak, 2012). Below is the flat-topped bulge of highly disintegrated rhyolite material, with minimal soil development, apparently a deposit produced by the slope collapse (Fig. 3a). Further down, rather subdued hummocky terrain with minor swells and hollows indicates an older phase of movement with this confined to the outcrops of sedimentary rocks emerging from beneath the rhyolites.
Fig. 3
Landslide slopes studied, with the location of sampled trees and reference sites: a)Suchawa site, b) Turzyna site, c) Garbatka site
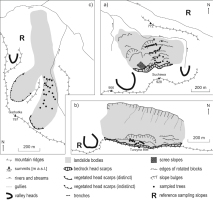
The morphology of the eastern side (Fig. 3a) of the complex suggests a different mechanism of movement. A series of benches elongated across the slope, with inclinations varying from 3–15° to 25–40°, is consistent with multiple rotational sliding of rigid rhyolite blocks on the deformable sedimentary substratum. As many as five rotated blocks occur along some slope transects. The area selected for dendrochronological study is located within the eastern part of the Suchawa landslide complex, while the reference slope is located on the opposite side of the Sokołowiec valley, on a steep but otherwise smooth slope, with no signs of deep-seated displacements.
Turzyna Landslide
Mt Turzyna (898 m a.s.l.) is one of the local peaks formed from a trachyandesite sill intruded into sedimentary rocks. The sill dips to the south with this accounting for the overall asymmetry of the ridge, with a low angle (less than 10°) southern slope and a very steep (30–50°) northern slope (Fig. 3b). The northern slope of Mt Turzyna terminates in a basal convexity at 690–720 m a.s.l., dissected by a series of parallel gullies each a few metres deep. The most conspicuous geomorphic feature of Mt Turzyna is a ridge-parallel bench near the summit, backed by a north-facing trachyandesite scarp up to 10 m high. The bench itself is 30–40 m wide and essentially flat, extending for nearly 400 m across the slope. The slope below the bench lacks a clear pattern of minor landforms and is rather monotonous, with occasional outcrops of jointed trachyandesite. Towards the east, the nearsummit scarp increases in height, to 20 m, and the bench disappears, whereas a poorly defined series of terrain steps occurs below.
An initial interpretation of the northern slopes of Mt Turzyna as landslide-affected (Berg et al., 1910) was confirmed by Bossowski et al. (1995) and Synowiec (2003). According to the latter author, the landslide at Mt Turzyna is in an early stage of displacement and no disintegration of the hillslope into a series of rotated blocks. By contrast, the section of the slope further east shows a relict landslide morphology, with a subdued step-like cross-section resulting from multiple sliding (Fig. 3b).
A dendrogeomorphological study was carried out on the western side of the landslide-affected slopes of Mt Turzyna, within the section of the landslide interpreted as being at an early stage of development.
Garbatka Landslide
The landslide terrain on the northern slopes of Mt Garbatka (797 m a.s.l.) differs from those previously described in terms of surface morphology and, apparently, origin (Fig. 3c). Here, the bulk of mass movement occurred within a broad valley floor and the most likely mechanism was a flowslide initiated by a shallow translational slide at the valley head which left a shallow slope hollow (Migoń et al., 2014). Thus, the valley floor is filled by a colluvial package with numerous floating boulders probably up to 10 m thick on the proximal side, thinning to a few metres in the distal, fan-like side. The mean inclination is ca. 10°. The total length of the landslide, from the head scarp to the most distant boulders, is nearly 1 km. Bedrock is composed of rhyolitic tuffs. Sedimentary rocks occur at the outlet of the valley and have been marginally involved in mass displacements.
Subdued surface morphology, the absence of any distinct toe, and the presence of well developed soils within the landslide terrain suggest a considerable age of the main phase of mass movement on the slopes of Mt Garbatka (Migoń et al., 2014). Whether the landslide is entirely relict or shows signs of instability is debatable. To this end, a portion of the colluvial fill at the upper end of the valley was selected for the dendrogeomorphological study.
. Materials And Methods
In order to conduct dendrochronological studies we sampled 140 cores from 70 Norway spruce trees, with two samples taken from each tree using a Pressler borer. Cores were taken at breast height along an axis parallel to the slope inclination or along the main axis of the stem (where there was evident eccentricity). This procedure enabled us to obtain tree-ring data from the upslope and downslope sides of each stem.
Only trees without visible loss in assimilation apparatus or stem injuries were sampled. There were 36 trees sampled on the Suchawa landslide, 13 on the Turzyna landslide, and 21 on the Garbatka landslide (Fig. 3). Since at each site we cored all trees suitable for dendrochronological sampling, the populations from each locality are dissimilar. The cores obtained were glued onto wooden holders and polished using sanding paper grades 100, 280, 500 and 1000, in sequence. Once the ring structures were clear, the tree-ring widths in each core were measured to 0.01 mm precision using LinTab measuring equipment with TSAPWin Professional 4.65 software. Skeleton plotting was used to reveal missing and wedging rings.
In addition, 60 samples from 30 Norway spruce trees were cored at three reference sites, 10 trees per site (Fig. 3). Reference sites were selected near the landslideaffected slopes, following the principle that environmental conditions (altitude, slope inclination, aspect, bedrock) are likely to be closely similar to the landslide slopes, yet without any geomorphic mass movement signatures, whether contemporary or relict. Reference cores were sampled and analysed in the same way as the cores taken from the landslide terrains.
The annual ring widths from each tree measured and their up and down-slope ring widths compared: percent eccentricity index and its yearly variations were calculated, based on the formula given in Fig. 4. Comparison with the samples from the reference sites allowed us to determine eccentricity threshold values, i.e. average levels of eccentricity recorded on stable slopes, unaffected by mass movement processes. In this way, we can eliminate the impact of non-geomorphic factors such as strong wind or snow creep from the further analysis of tree rings produced on landslide-affected slopes. Thus, if the value of the yearly variation index for a given year on the landslide site fell outside the range of the eccentricity recorded at the reference site, ground disturbance due to downslope movement was assumed as the most likely reason for an eccentricity ‘event’. Episodes of landslide activity are therefore revealed in the tree ring record as abrupt increases or decreases in index values, but are interpreted as such only if the tree-ring reaction is stronger than the reference threshold value. The strength of a landslide event is defined by the mean value of the yearly variation index for all trees assumed to record ground instability, i.e. for those showing index values above the threshold value. Detailed assumptions of the method and examples of its application are presented by Malik and Wistuba (2012) and Wistuba et al. (2013). An example of transformation of the raw tree-ring widths into an eccentricity index and its yearly variation index, with an example of landslide movement identification, are shown in Fig. 4.
Fig. 4
Example showing use of tree-ring eccentricity index in detecting landslide activity at Garbatka as applied to two cores sampled from a single spruce tree: a) measured tree-ring widths, b) calculated values of eccentricity index, c) yearly variation of eccentricity index, d) landsliding events dated. U — width of tree ring in the upslope part of stem (mm); D — width of tree ring in the downslope part of stem (mm); E — eccentricity of tree ring (mm); Ei — eccentricity index of tree ring (%); vEi — yearly variation of eccentricity index (%); x — year (annual tree ring).
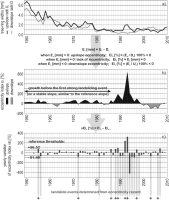
Finally, the results of dendrochronological dating were compared with the rainfall record from Mieroszów weather station during the growing seasons (April to September), 1977–2007. To describe the relationship between dendrochronological events, interpreted as resultant from ground displacement, and precipitation record correlation coefficients have been calculated for data sets describing the number of eccentricity ‘events’ in each year in 1977–2007 — Fig. 5b, Fig. 6b, Fig. 7b) and precipitation pattern (for annual and April-September totals; Fig. 5a, Fig. 6a, Fig. 7a). Six coefficients, two for each study site, were obtained.
Fig. 5
Dendrochronological reconstruction of activity on the Suchawa landslide: a) April-September precipitation totals, b) dendrochronological events, c) up and downslope events, d) strength of dendrochronological events.
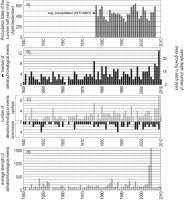
. Results
Temporal Coverage And Threshold Values
The oldest trees were sampled from the Suchawa landslide, trees from the other two landslide localities are younger. Thus, the following periods are fully covered by tree-ring data: 1944–2010 on the Suchawa landslide, 1952–2011 on the Turzyna landslide and 1952–2010 on the Garbatka landslide (Table 1). Dendrochronological data for the 70-year long period 1940–2010 were subjected to further analysis.
Table 1
Sample numbers, age of the oldest tree, the youngest tree and average age of trees sampled on all sites studied.
Threshold values for the yearly variation index were separately established for each locality, based on data from the reference slopes. At the Suchawa landslide values for upslope eccentricity >59.39% and for downslope eccentricity <–64.35% were considered as evidence of ground instability. At the Turzyna landslide the respective values are >58.96% for upslope eccentricity and <–64.86% for downslope eccentricity. Finally, at the Garbatka landslide an upslope eccentricity value of >56.52% and downslope eccentricity value of <–51.40% were included for further analysis. The lower threshold values at the Garbatka site reflect the less inclined slopes compared with the other two sites.
Suchawa Landslide
Within the 1940–2010 period, 36 trees showed 262 disturbance eccentricity ‘events’, with upslope eccentricity predominating (68%, Fig. 5c). The number of trees affected varied from year to year. In certain years nearly 20 per cent of the trees were affected, although in most years the percentage did not exceed 15% (Fig. 8b). The highest value was noted in 2009 (reaction of 13 trees, i.e. 40.8% of sampled population), followed by 1976, 1982,1988, 2004, 2007, and 2008 (reaction of at least six trees in a year, i.e. at least 16.2%). The strongest average reaction were recorded in 2004 and 2005 (Fig. 5d) and only in two years trees showed no eccentricity ‘events’.
Turzyna Landslide
At the Turzyna landslide site, 121 eccentricity ‘events’ were recognized in the dataset. As in the previous case, the dominance of upslope eccentricity is evident (88%, Fig. 6c). While in 11 years within the 1940–2011 record no instances of growth disturbance above the predetermined threshold values were found, the percentage of trees affected is higher than at the Suchawa site. In certain years nearly 50 per cent of trees recorded eccentricity ‘events’ with the relatively large percentages characterizing the years 1960, 1974, 1976, 1978, 1982, 1984, 1996, and 2007, with at least four (i.e. 30%) of the trees showing growth disturbance (Fig. 6b, Fig. 8c). However, the size of the sample (13 trees in total) is distinctively smaller than at Mt Suchawa. The strongest average reaction of trees was recorded in 1952, 1976, 1985–1986, and 2007 (Fig. 6d).
Garbatka Landslide
At the Garbatka locality (N = 21), 140 instances of growth disturbance in the form of anomalously high eccentricity were recorded, with upslope eccentricity dominating (71%, Fig. 7c). There was no evidence of any above-threshold disturbance in the 16 years out of 70 covered by the record. The highest frequency of eccentricity ‘events’ was noted in 1989 (six trees, i.e. 28.6% of the total), followed by 1965, 1977, 1979, 1984, 1997 (reaction of five trees each year) (Fig. 7b, Fig. 8d). The strongest average reactions were recorded in 1983, 1992 and 1993 (Fig. 7d).
. Discussion
Deciphering The Nature Of Ground Movement
At each of the three landslides eccentricity ‘events’ during the last 70 years have been frequent and have occurred almost every year. Among them, upslope eccentricity clearly predominates (68–88%), meaning that tree stems tend to lean upslope, parallel to the slope. Malik and Wistuba (2012) found that such a pattern of stem behaviour is typical for slopes formed by landsliding and, by inference, ground deformation revealed by growth eccentricity in the Kamienne Mountains is interpreted as the consequence of ongoing sliding along a shear surface rather than an effect of superficial processes such as soil creep, flow, or surface wash acting on the stable surface of an old landslide.
Despite the predominance of up-slope eccentricity, there is a notable difference between the Turzyna site and the other two. At Turzyna the percentage of trees leaning downslope is markedly lower (12%) than at the two other sites (29 and 32%), probably due to the morphology of the landslide terrain. At Mt Turzyna, sampled trees grow on the subsided block whose upper surface is sloping north at a very low angle (landslide at an early stage of development according to Synowiec (2003), whereas at the other two sites the terrain is steeper and the relief is more complex. At the Suchawa landslide in particular, flat benches and steep scarps alternate and it is on the latter, sloping by as much as 30° or more, where downslope tree tilting is common. This pattern is consistent with the findings of Braam et al. (1987) who highlighted a connection between local hillslope morphology, style of stem deformation and character of tree ring eccentricity.
The rates of displacement recorded by dendrochronological events is likely to be low. Since neither historical accounts nor geomorphic evidence suggest any acceleration of mass movement within the landslide terrain in the Kamienne Mountains in recent times. Possible analogues are slow-moving landslides, where the mean annual rate of movement is from less than 1 mm (Klimeš et al., 2012), to centimetres or tens of centimetres (Noferini et al., 2007; Fernández-Merodo et al., 2014; Klimeš et al., 2012; Handwerger et al., 2013; Grana and Tommasi, 2014), possibly up to 1–1.5 m (Handwerger et al., 2013; De Vita et al., 2013; García-Davalillo et al., 2014). In these cases, slight tilting and bending of stems, similar to that recognized in the study area, are the only visible manifestations of ground deformation (e.g. Fig. 1b; compare with Fig. 1a — during abrupt catastrophic landslide events trees usually fall down or are strongly inclined). Whether these low displacement rates are precursors of a phase of faster movement, as described by Massey et al., (2013) in the Spanish Pyrenees or by Massey et al., (2013) in New Zealand, cannot be satisfactorily resolved.
Temporal Variability Of Landsliding
Dendrochronological events of landsliding at the three sites, show little between-site relationship (Fig. 8). Nevertheless, there are years and sub-decadal intervals, during which a relatively high frequency of events occur suggesting ground displacement at a larger scale, involving more extensive areas.
Prior to 1977, since the rainfall record began, common elevated levels of geomorphic activity are only evident in 1965–1966, at the Suchawa and Garbatka sites. At the former site, further years of apparently increasing activity are 1955 and 1976 and at Mt Garbatka 1970– 1972. In subsequent years, 1974–1984) stand out at all localities, as well as 1995–1998, although this is rather poorly visible in the record from Mt Turzyna. Isolated years of enhanced activity, although with no parallels at other localities, are 1986 and 1988 at Mt Suchawa and 1989 at Mt Garbatka. In recent times, the interval 2006– 2009 is again characterized by a higher frequency of growth disturbance events but individual records for separate sites do not match (Fig. 8). However, at Mt Turzyna both the sample size (N = 13) and the sample area are small and therefore the evidence for slope movement should be interpreted with caution.
Triggering Factors For Landslide Activity
Comparison of the rainfall records with the dendrochronological record for the three landslides reveals that in years of increasing activity correspond with higher than average April to September precipitation amounts (Figs. 5, 6, 7). At the Suchawa landslide such coincidences occurred in 1980, 1995, 1998, and 2007, whereas at the Turzyna landslide activity years are 1984, 1996, and 2007. However, other years or periods of high April-September precipitation such as 1977, 2001, and 2005– 2006 are uncorrelated with an increasing frequency of dendrochronological events. Therefore, for the entire period covered by the rainfall record the comparison of dendrochronological results from the Turzyna and Suchawa landslides with April-September precipitation shows very poor correlation (0.03, 0.04 respectively: Table 2).
Table 2
Correlation coefficients for activity of Suchawa, Turzyna and Garbatka landslides and precipitation 1977–2007 (yearly totals and April to September totals)
Suchawa landslide | Turzyna landslide | Garbatka landslide | |
---|---|---|---|
annual precipitation total | 0.11 | 0.18 | 0.25 |
precipitation totals April-September | –0.03 | 0.04 | 0.53 |
Elsewhere, deep-seated rotational landslides, similar to studied localities, have been coupled with long-term seasonal rainfall patterns (> 30 days) (e.g.Zêzere et al., 2005), hence a better correlation could be expected at Suchawa and Turzyna. The reasons for the poor match between the records may be related to specific lithological conditions in the area. Both rhyolites at Mt Suchawa and trachyandesites at Mt Turzyna are heavily jointed and thus have high secondary porosity. Visual confirmation of high bedrock permeability is shown by the low density of perennial streams and a large number of dry valleys; it is also reflected in local toponyms such as the name of this section of the Kamienne Mountains itself (Suche Mts= ‘Dry’ Mountains). Here, rainwater easily drains through open cracks and joints, and along the deep-seated shear surfaces, emerging on the lower slope underlain by sedimentary formations, not necessarily causing ground movement. Alternatively in a few cases there is a 1–2 years lag between high rainfall and the increasing frequency of events recorded by tree growth (Fig. 5). For example, the ‘disturbance year’ of 1982 followed high rainfall in 1980, one in 1998 followed a wet period of 1995–97, whereas one in 2004 was preceded by two wet years in 2001–2002. This temporal relationship suggests a link between long-term groundwater storage and delayed reactivation of landslides.
By contrast, on the gentler slopes of the Garbatka landslide the correlation between the number of dendrochronological events and seasonal precipitation is much higher (0.53) (Table 2). Thus, all four years on record with five events recorded (1977, 1979, 1984, 1997) correspond with spring-summer rainfall above the long-term average (Fig. 8). Furthermore, four out of six years typified by the number of events n = 4 match rainy years. However, the year with most slope activity (1989) was not particularly wet
In terms of origin and mechanism of movement the landslide at Mt Garbatka is significantly different from the other two examples. The landform assemblage indicates that the primary mechanism was a flowslide and the geophysical survey showed that the basal detachment surface is at most 10 m below the surface (Migoń et al., 2014) and furthermore, the landslide body occupies a valley floor which tends to receive additional water from the surrounding slopes. However, although the landslide itself originates in porous rhyolitic tuffs and the top part of colluvial materials (up to 2 m) often show an openwork structure, favourable conditions for waterlogging and pore-pressure changes at greater depth may occur.
Shallow landslides, like Garbatka slide, according to Zêzere et al. (2005) may have shorter response times to rainfall, in the order of 1–15 days, but they do not necessarily move fast. Precipitation and resultant hydrological/hydrogeological conditions are often mentioned among factors triggering and controlling the slow motion of landslides (Macfarlane, 2009; Fernández-Merodo et al., 2014; Comegna et al., 2013; García-Davalillo et al., 2014). According to Handwerger et al. (2013), precipitation drives seasonal velocity changes in slow-moving landslides by increasing pore-water pressure. This factor was also pointed out as decisive by Ranalli et al., (2010) and Massey et al., (2013). According to the latter authors, slow landsliding is strictly connected to changes in the groundwater table. Pore-water pressure plays a major part, regardless of the size and thickness of a landslide, and landslide activity appears sensitive to even subtle hydrologic perturbations (Handwerger et al., 2013). According to Massey et al., (2013), brief episodes of faster displacements are triggered by seasonal peaks in pore pressure, linked to long periods (12–20 weeks) of increased precipitation and reduced evapotranspiration. Macfarlane, (2009) on the other hand found that higher rates of movement in slow landslides occur when the cumulative rainfall over a period of 3–4 months exceeds 300 mm. Suitable precipitation and hydrogeological conditions make rates of movement increase over a period of months and then gradually diminish (Macfarlane, 2009).
There are further differences between the nature of dendrochronological events at the study sites, consistent with the different movement mechanisms and their relationships with rainfall patterns. Trees growing on the Garbatka landslide record ground instability through large, sudden jumps (increases for upslope and decreases for downslope tilting) in the value of eccentricity index. Later on, index values return to the previous low level over similarly brief periods (Fig. 4b). These jumps at the Garbatka landslide can be interpreted as episodic activity typified by short-term displacement accelerations, abruptly suppressed afterwards. The opposite patterns are identified for the Suchawa and Turzyna landslides where high levels of eccentricity lasting for longer, suggesting slow but more continuous movement.
The above analysis provides a basis for an attempt to infer the amount of seasonal rainfall in the period prior to the opening of the Mieroszów precipitation gauging station. Higher frequencies of tree growth disturbance ‘events’ at the Garbatka landslide were recorded in 1965 and 1970–72, suggesting rainfall higher than average in these years. Poor correlation between dendrochronological events and precipitation at the other two sites does not really allow for similar inferences. Nevertheless, it is notable that both the Suchawa and Turzyna landslides clearly showed an increasing level of activity in 1976, as well as in the consecutive years 1954–55 and 1965–66. However, the dendrochronological record from the Garbatka landslide does not show any corresponding increasing disturbance.
While analysing the relationships between dendrochronological events of landslides and the rainfall totals one should not overlook limitations imposed by the nature of the rainfall record itself. First, only monthly totals were available and possible episodic cloudbursts cannot be inferred from the dataset. While it is unlikely that cloudbursts would have had a significant effect on deepseated landslides, their effect on the shallow Garbatka flowslide would remain undetected even if present. Second, although the Mieroszów station is close to the landslide sites, it is nevertheless 4 km from Garbatka, 6 km from Suchawa and 8 km from Turzyna. Starkel (2012) has recently showed the sensitivity of rainfall records to increasing distance from sites of maximum rainfall during a cloudburst in a mountain area. Third, there may be an effect of altitude and topography in that the landslide sites are 150–300 m upslope, within a mountain range, compared with the town of Mieroszów located in an intramontane basin.
Precipitation is not the only known trigger of landsliding. Seismic activity is another mechanism for landslide initiation and reactivation (Keefer, 1984; Koi et al., 2008; Lin et al., 2008; Schulz et al., 2012). Recent research by Klimeš et al. (2012) and Fotopoulou and Pitilakis (2013) has shown that earthquakes can accelerate slow-moving landslides by a factor of ten. In the last decades and centuries earthquakes with maximal macroseismic magnitudes of M<6 were recorded in southern Poland (Guterch, 2009). In the last 70 years earthquakes with M≥2.9 occurred in 1942, 1966, 1992, 1993, 1994, 1995, 2004,2005) (Guterch, 2009), but their possible influence on the activity of landslides has yet to be explored. Dendrochronological studies in the Flysch Carpathians (Krąpiec and Margielewski, 1991,Krąpiec and Margielewski, 2000; Krąpiec et al., 2008) show that slow sliding may occur as a result of minor earthquakes, and landsliding due to seismic tremors was suspected in the Beskid Niski Mts, 100 km from earthquake epicentres. In the Sudetes, earthquakes are weaker than in the Flysch Carpathians (magnitudes are less than 3; Guterch, 2009). Yet, since the 16th century 23 earthquakes were reported from the area of the Sudetes and the Sudetic Foreland, among them a well documented earth-quake in 1895 with the reconstructed magnitude of 4.5 (Guterch, 2009). Although Ciężkowski and Koszela (1988) linked the historical rockslide in Bardo (Central Sudetes) with an earthquake in 1598, the proposal remains highly speculative, particularly given the temporal coincidence of the landslide with high precipitation and direct slope undercutting during the resultant flood (Migoń et al., 2002). Also according to Jibson (2012) earthquakes with a magnitude below 4.0 are unlikely to produce slope instability but it depend on slope angle and type of rock material which compose the slope. Therefore, it is hard to tell if small earthquakes in the Sudetes can trigger landsliding.
. Conclusions
Dendrochronological studies suggest that the three landslides under study, despite their apparent stability and the absence of any historical records of activity, remain active and currently belong to a group of slow-moving landslides. The morphology of slopes is transformed by continuous minor sliding, recorded by trees almost every year. Slope material is transported at slow rates and most probably over very short distances.
Ground displacements are recorded mostly by upslope eccentricity developed in spruce stems tilted upslope, with this especially well visible on the deep-seated Turzyna site where rotational sliding is at an early stage and trees are mostly tilted upslope. Where landslide relief is better developed, on the studied slopes of Mt Suchawa and Mt Garbatka, relatively more trees are tilted downslope.
Slow landsliding occurs more or less synchronously on all three studied slopes, although with variable intensity. In certain years it affects more extensive parts of slopes, but more often ground movement occurs locally, in limited areas and at different intensities.
Correlation of the dendrochronological record with the rainfall record from a nearby station in Mieroszów 1977–2007 is very poor for two deep-seated rotational slides at Mt Suchawa and Mt Turzyna but considerably better for the shallow flowslide at Mt Garbatka. This may reflect higher permeability of heavily jointed rocks involved in deep-seated sliding but may also be linked to imperfections in the rainfall record itself. The temporal coincidence of dendrochronological events and higher rainfall at Mt Garbatka is maybe consistent with the flowslide origin of the landslide and indicates shorter response times to atmospheric events.
The dendrochronological method of tree-ring eccentricity analysis applied in this paper appears to be an efficient tool in detecting minor landslide movements. It is possible that the approach would also prove efficient in detecting minor, preparatory movements which might precede catastrophic events and as such, would become a component of early warning system. Therefore, it should be an object of further studies.