. Introduction
In our studies, we used tree-rings as indirect archives of climate changes and environmental pollution in 20th and 21st century. In Poland the highest levels of pollutants were recorded in 1980s (Boden et al., 2016). Since the 1980s, a few environmental projects have been implemented in most factories in Poland, similarly to many countries in Europe. Since the 1990s a decrease of pollutants emission has been noted. The observed anthropogenic impact on the environment causes changes in the elemental composition of the atmosphere and the biosphere. Trees intercept and absorb air from the atmosphere and they can be used as sensitive bio-indicators of ecosystem changes. Scots pine has been used in biomonitoring studies, because this species is sensitive to climate and the anthropogenic effect (Schweingruber, 1996; De Vries et al., 2000; Sensuła et al., 2011a, 2011b; Sensuła and Pazdur, 2013a, 2013b). Scientific literature reviews show, that the industrial pollutants usually have a negative impact on vitality and annual radial growth of trees. The strength of the negative impact of pollution on trees can be varied in space and depends on the habitat conditions and distance from the source of pollution, directions of prevailing winds (Wilczyński, 2006; Danek, 2007; Elling et al., 2009; Malik et al., 2012). The trees, growing under stress of the pollutants, reduce their radial growth and height growth (Kienast, 1985, Schweingruber, 1986; Krąpiec and Szychowska-Krapiec, 2001; Danek, 2007; Elling et al., 2009; Malik et al., 2012; Crecente-Campo et al., 2010). Also the differences in the similarity of radial growth response of pines to individual climate factors (temperature, sunshine, precipitation, air humidity) has been observed (for example Sensula et al., 2015a, 2015b).
The climate changes and industrial pollutant emissions occur during physiological processes responsible for plant growth and also can influence stable isotope composition of wood and its components (Craig, 1954; Farquhar and Lloyd, 1993; McCarroll and Loader, 2004; Sensuła, 2015). There has been much discussion about the biological effects of pollutant pressure on trees, which concerned yearly variation of stable isotope concentration in tree rings and needles (Craig, 1954; Farquhar and Lloyd, 1993; Field et al., 1995; McCarroll and Loader, 2004; Pazdur et al., 2007, 2013; Keeling et al., 2010; Parn and Madre, 2011; Sensuła and Pazdur 2013a, 2013b; Battipaglia et al., 2014; Saurer et al., 2014; Sensuła, 2016a, 2016b). The isotopic records in tree-rings are sensitive bio-indicators of the way that the components of air and water have been changed by the trees in response to the environments in which they are grown (Craig, 1954; Farquhar and Lloyd, 1993; McCarroll and Loader, 2004; Sensuła, 2015). It is possible to estimate from the isotope response, whether a plant reacts more strongly on the supply side (stomata) or the demand side (biochemical) of photosynthesis (Scheidegger et al., 2000; Farquhar and Lloyd, 1993). Previous studies have shown changes in climate signal in stable isotope composition of α-cellulose of tree ring cellulose as well as deviant trends between δ13C and δ18O series caused by pollution in different parts of Europe during the 20th century (Boettger et al., 2014; Sensuła, 2016a).
According to the NOAA (2016), the average global atmospheric CO2 concentration has risen from 331 ppm in 1975 to 393 ppm in 2012. The observed anthropogenic impact on the carbon cycle is mainly related to various global industrial activities (Boden et al., 2016) that have caused changes in the isotopic composition of carbon in global air atmosphere (Martin et al., 1988, Ferrio et al., 2003; Keeling et al., 2010; Pazdur et al., 2013). Elevated CO2 significantly decreases δ13C in the air (Fig. 1). The δ13C present in air is about -8.3‰. A depletion of δ13C values may be due to the global and regional effects of fossil fuel burning and it may be also due to emissions connected with local sources of pollution (inter alia industrial factories, vehicles, low stack emission of CO2).
Fig. 1
Decrease of δ13C in the air due to increase of CO2 (according to Boden et al., 2016; McCarroll et al., 2009).
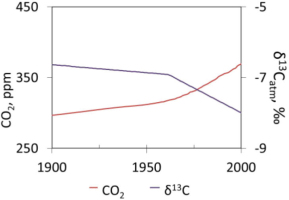
The aim of our studies was to investigate the sensitivity of the pine populations to various climatic factors (such as the air temperature, the precipitation, the humidity and the sunshine duration) in the area influenced by the industrial pollution. The variation of the annual radial growth was used as an indicator of the tree’s response to climate factors during the period of time since 1951–2012, when strong increase of industrial activities in the investigated area were observed. The sampling sites were located in different distance and in different geographical directions from industrial factories (Sensuła et al., 2015a, 2015b).
The mass spectrometric analysis of the carbon and oxygen stable isotope composition of cellulose extracted from the pine population growing in the most polluted site was complementary to dendrochronological studies. The isotopic composition of pine tree-rings was used to study the climatic changes in the industrialized area in the past to better understand future consequences of ecosystem changes (Schweingruber, 1996; De Vries et al., 2000; McCarroll and Loader, 2004; Pazdur et al., 2007, 2013; Sensuła et al., 2011a, 2011b; Sensuła and Pazdur, 2013a, 2013b; Sensuła, 2015). Combined measurement of carbon and oxygen isotopes ratio may give information about the changes in stomata conductance (gs) and changes in photosynthetic capacity (Amax – the average maximum net photosynthesis at ambient CO2 concentration under optimal environmental conditions) and indicate which factor responded more strongly (Farquhar and Lloyd, 1993; Scheidegger et al., 2000) to variation in the growth environment. According to Farquhar and Lloyd (1993) these changes can be also described by carbon isotope discrimination (Δ13C), which may be considered as a photosynthesis-weighted integrator of carbon supply and demand.
The objectives of this study were to analyze: (1) the record of climate changes and (2) the biological adaptation to pollution of pine growing nearby chemical and nitrogen factories in Kędzierzyn-Koźle during the period of industrial development and implementation of proecological policy in Poland. There is still a lack of data concerning the environmental changes in the most industrialized part of Poland over the last century. In these studies, we determined the stable carbon and oxygen isotopes concentration in annual tree rings of pine growing in close proximity to chemical factories in Kędzierzyn-Koźle during the period of industrial development and implementation of pro-ecological policy in Poland.
. Materials and methods
Research area
Kędzierzyn-Koźle (50°20 ′N; 18°13′E) is located near two factory complexes (Fig. 3): Blachownia Holding S.A. (previously named: Blachownia Chemical Factory), where chemical production began in 1941, and nitrogen factory Grupa Azoty ZAK (previously named: Zakłady Azotowe Kędzierzyn-Koźle, ZAK) where production started in 1954. In both factories, many different units and production facilities were constructed and added over a period of the last 60 years. Since the1980s, numerous projects dedicated to environmental protection were implemented in both factories. After many years of trying to minimize the negative impact of production processes on the environment, these factories were removed from the list of the most environmentally noxious companies. In 2004, the Waste Management Plan for the City of Kędzierzyn-Koźle set strategic objectives for 2015, inter alia: the introduction of the principles of “cleaner production”. The companies operating in Kędzierzyn-Koźle are of strategic importance to the region and the country.
Meteorological data
The period from 1951 to 2012 AD was characterised in the regional climate records by an annual average temperature of about 9°C (data range from 6.7 to 10.6°C), and a mean annual sum of precipitation of around 610 mm (data range from 359 to 868 mm/year); mean annual number of sunshine hours is approx. 1530 (data range from 1108 to 1978), relative humidity around 80% (data range from 75 to 86%). The lowest precipitation was observed between mid-1980s and mid-1990s (Fig. 2). The vegetative period begins in April and lasts until September. The meteorological data were obtained thanks to the Polish Institute of Meteorology and Water Management (IMGW-PIB). Temperature, humidity and precipitation data comes from the meteorological station in Opole, whereas sunshine data come from the meteorological station in Katowice.
Samples and standardization process
Scots pine (Pinus sylvestris L.) is a dominant species in the investigated area. For dendrochronological analysis, twenty trees were selected from each population (tree stands). The examined pines were healthy and grew in similar habitat conditions at different distances and directions from the industrial factories (Fig. 3).
All sites were classified as fresh mixed broadleaved forests. In order to avoid different dendroecological reaction of juvenile wood, an attempt was made to select pine stands aged between 80 (site A) and 100 years (sites C, B, N, S and T) which is the felling age of Scots pine. The pine trees were dominant or co-dominant individuals.
One increment core from each tree was taken at a height of 1.3 m above ground. Tree-ring widths were measured to the nearest 0.01 mm. The tree-rings were dated and rechecked using the COFECHA program (Holmes, 1983). Each tree-ring width series was standardized to remove non-climatic trends due to age and growth effects. Therefore, in each year the annual sensitivity index (si) was calculated according to the formula:
where xi is the tree-ring width in year i (Douglas, 1920).
The mean change of the tree-ring width for the 1951–2012 period was evaluated by the mean sensitivity (MS). MS indicates interannual changes of the trees’ sensitivity to climate factors (Fritts, 1976). The site sensitivity chronology was constructed on the basis of series sensitivity. The site sensitivity chronology exposed the short-term variance due to variation of climatic factors. The similarity of short-term incremental reactions of trees in each pine populations was evaluated by calculating the mean between-tree correlation (rbt). The calculated index EPS enables an assessment of the representativeness of the constructed site chronologies (Wigley et al., 1984).
Ordination of the pine populations and climate-growth relationship
The principal component analysis (PCA) was applied to identify the short-time factors affecting tree-ring widths. The identification of PC1 and PC2 was based on an analysis of the component scores. The variables (n=52) were site sensitivity chronologies. The cluster analysis (CA) based on Ward’s method and 1-r Pearson’s distance has been used to analyse similarity of the response of each pine population to climate elements in the 1951–2012 period. The variables (n=52) were correlation coefficients between the site sensitivity chronologies and monthly temperature, precipitation, relative humidity and sunshine duration from the prior September to the current September. Response function analysis (Fritts, 1976; Holmes and Lough, 1999) was used to identify the climatic factors that determined the PC1 and PC2. These results were verified by analyzing the correlation between site sensitivity chronologies and the climate parameters.
Isotopic measurements
The analysed samples covered the period 1975–2012 AD. The α-cellulose samples were extracted from increment cores of ten representative trees growing in the C sampling site. The pines growing in this sampling site were characterized by the highest level of tree ring width reduction in the period of time between 1958–1991.
The absolutely dated annual tree-rings were manually separated as thin slivers and pooled and homogenized. The α-cellulose samples were extracted by applying the procedures based on the Green’s method (1963) used in The Mass Spectrometry Laboratory of the Silesian University of Technology (Pazdur et al., 2007, 2013; Sensuła et al., 2011a, 2011b; Sensuła and Pazdur, 2013a, 2013b). This method includes the following steps: the removal of lignin, the processing of the holocellulose to α-cellulose, bleaching, neutralizing and drying (Green, 1963).
The initial stage of the process involved cutting of annual rings from the cores in the sample producing fine shavings <0.5 mm thick, cut from wood with a knife. Afterwards, the samples were treated with a toluene-ethyl alcohol mixture (in proportion 1:1, at 80°C, over 4 hours), ethyl alcohol (at 80°C, over 4 hours), distilled water (at 80°C, over 1 hour). The reaction was performed in a Soxhlet apparatus. Afterwards, the samples were dried overnight. The dried and weighed samples were placed into the glass test-tubes. The next step was bleaching with NaClO2 and CH3COOH solution (175 ml of distilled water, 2.5 g of NaClO2 and 1.7 ml of CH3COOH was added per 1g of each sample) at 70°C, over 1 hour in order to remove lignin and to extract cellulose. This process was repeated 5 times. In the next step the solution was removed by decanting. The samples were rinsed with boiling distilled water and then with cold distilled water up to neutral pH. Afterwards, the samples were treated with 50 ml of 10% NaOH solution (at 70°C, over 45 minutes). Afterwards the solution was removed and samples were rinsed with cold distilled water. Then the samples were treated with 17% NaOH solution (at room temperature for 45 minutes). The solution was then removed from the tubes and samples were rinsed with distilled water. At the end of the extraction process the samples were treated with 10 ml of 1% HCl solution and rinsed up to neutral pH. The obtained α-cellulose was dried on a hot plate at 60°C overnight. To ensure homogeneity the chemical pre-treatment was carried in an ultrasonic bath.
In order to determine the δ13C values, the samples (0.060 mg) were loaded into tin capsules and combusted at a temperature of 1100°C, CO2 was separated in a gas chromatography column of the elemental analyser (EuroVector). In order to determine the δ18O values, the samples (0.095 mg) were loaded into silver capsules. To displace moisture-containing air in the cellulose samples, the samples were heated over 24 hours in a vacuum line (60°C) and after that they were put into a special air-filled dry box before stable isotope ratio determination (Sensuła et al., 2011b). The samples were converted to CO by pyrolysis at a temperature of 1350°C and separated in a gas chromatography column in the elemental analyser (EuroVector).
The stable oxygen and carbon isotope compositions of the samples were determined using an Isoprime continuous flow isotope ratio mass spectrometer (GV Instruments, Manchester, UK) at the Mass Spectrometry Laboratory of the Silesian University of Technology.
The relative deviation of the isotopic composition is expressed, in parts per thousand (‰), as δ=(Rsample /Rstandard −1)⋅ 1000, Where Rsample and Rstandard are the ratios of the heavy to the light isotope concentration in the sample and in the standard, respectively. The δ13C results are reported in values relative to VPDB (Vienna Pee Dee Belemnite), whereas the δ18O results are reported in values relative to VSMOW (Vienna Standard Mean Ocean Water). In these measurements, wood (C-5) and α-cellulose (C-3) reference materials from IAEA were used.
The isotopic discrimination in photosynthesis was calculated according to the model of Farquhar and Lloyd (1993):
where Ci is intercellular CO2 concentration, Ca is ambient CO2 concentration, a (ca. 4.4‰) is the discrimination against 13CO2 during CO2 diffusion through stomata, b (ca. 27‰) is the discrimination associated with carboxylation.
. Results
Each of the pine chronologies constructed in this study is a local growth pattern of the pine population. These isotopic records are the result of the response of the trees to variations of various environmental factors: climatic drivers and human activities. For the statistical calculation, Statistica 12 software (StatSoft, Inc., 2014) was applied.
Tree-ring chronologies and dendrochronological indices
The average width of the radial increment of the studied pine populations ranged from 1.31 mm to 2.20 mm (Table 1). Tree-ring width chronologies, apart from their short-term variance are characterised by a clear long-term variance (Fig. 4). Chronologies of sensitivity display a strongly reduced long-term variance while the short-term variance is highlighted. The average sensitivity of pines for the period of 1950–2012 was included in a narrow range from 0.214 to 0.281, while the rbt index was between 0.399 and 0.521 (Table 1). On the other hand, in all cases the EPS considerably exceeded the minimum value of 0.85. These results indicate a relatively high sensitivity of the pines, the strength of the climatic signal and representativeness of site chronology. The similarity of year-to-year changes of the tree-ring width of 6 pine populations was relatively high. Tree-ring width chronologies also had a similar long-term course.
Fig. 4
The course of 6 sites tree-ring width chronologies (top) and sites sensitivity chronologies (bottom).
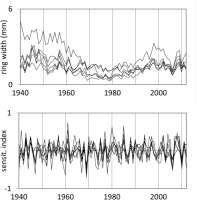
Table 1
Statistical characteristics of the site chronologies for the 1951–2012 period. TRW – mean tree-ring width; TRI – mean tree-ring index; MS – mean sensitivity; rbt – the mean correlation of standardized series of trees – the signal strength of the chronology, EPS – the expressed population signal is a statistic for examining the common variability in a chronology.
In the 1970s the pines strongly reduced their radial increments (Fig. 4). This was a result of the increase in industrial pollution at this time (Fig. 1). The reduction in tree rings radial growth in 2003 and 2006 were due to drought events
It is interesting that the year-to-year sensitivity of trees remained at a similar level throughout the analysed period (Fig. 4). The changes of tree-ring widths from year to year are the result of the impact of the climate on trees. Therefore, it can be assumed that the climate signal contained in the chronologies of pine populations was at a similar level in the analysed period.
Short-term changes of radial increments of the pine populations differed. This diversity is indicated by the location of the site sensitivity chronologies in relation to component loadings of PC1 and PC2 (Fig. 5). Sensitivity chronologies are strongly differentiated mainly by PC2. The A chronology positively correlates with PC2, while the correlation is negative in the case of S (Fig. 5). Other chronologies (B, C, N and T) show very poor correlation with PC2. They form a compact group and correlate strongly with PC1, while the chronology of populations A and S correlate poorly with PC1 (Fig. 5). The PC1 accounts for 78% of the variance among 6 sensitivity chronologies and the PC2 accounts for 10%. PC1 was most effective for explaining the variance of the radial growth of the pines.
Fig. 5
Comparison of the loadings of the first and second principal components for 6 sites (A, B, C, N, S, T, see Table 1) sensitivity chronologies.
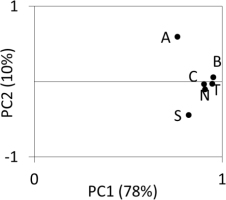
Climate determines the short-term variance of incremental reaction of trees. This kind of variance is illustrated by sensitivity chronologies. It can therefore be assumed that the two main components – PC1 and PC2 – depict the climate factors that had a significant impact on the variance of radial increments of pines. This is confirmed by the results of cluster analysis. It was found that a series of correlation coefficients for the populations B, C, N and T formed a single cluster, while the series of populations A and S differed significantly (Fig. 6).
Fig. 6
The dendrogram of clusters of pine population (A, B, C, N, S, T, see Table 1) based on the similarity of their response to climatic conditions. Localization of the pine population with similar response.
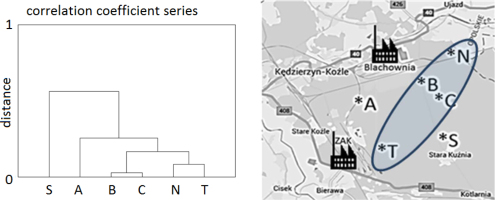
With the response function analysis, the climate features described by PC1 and PC2 were identified (Fig. 7). It turned out that PC1 correlated significantly (p<0.05) with monthly values of relative humidity and precipitation of September of the preceding year, humidity, temperature and sunshine duration of spring months and with rainfall, temperature, and sunshine duration of the summer months in the year of ring formation (Fig. 7). On the other hand, PC2 correlated significantly (p<0.05) with the humidity, sunshine duration, temperature and rainfall of spring and summer months of the current year, and in addition with the rainfall in January and temperatures of September and December of the previous year (Fig. 7).
Fig. 7
Significant (at p<0.05) regression (response function) coefficients calculated between PC1 (white bars), and PC2 (black dots) and monthly temperature (T), precipitation (P), sunshine duration (S) and relative humidity (H) from September of the previous year (pS) to September of the given year (S).
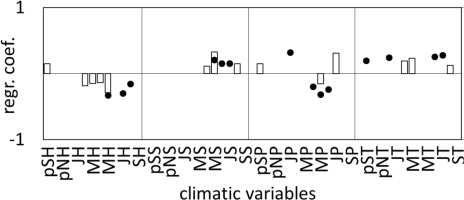
In 3 cases PC1 and PC2 were significantly correlated with the same climate parameters. This applies to relative humidity, rainfall and sunshine duration in May of the year of ring formation (Fig. 7). The impact of climatic conditions of this month on the radial increment of pines therefore requires thorough consideration. The principal component analysis showed that the climatic factors described by PC1 have a similar effect on the radial increment of 6 pine populations. In turn, climate elements described by PC2 were differentiated by the incremental rhythm in pine growth (see Fig. 5). The above results were verified by correlating these climate parameters with site sensitivity chronologies (Fig. 8). It turned out that the relation between a relative radial increment and the temperature of February, March and August were very similar in all populations (Fig. 8). These results correspond with the results obtained for PC1 (Fig. 8).
Fig. 8
Correlation coefficients between site (A, B, C, N, S, T, see Table 1) sensitivity chronologies and monthly climatic variables: temperature (T), precipitation (P), sunshine duration (S) and relative humidity (H) from September of the previous year (pS) to September of the given year (S).
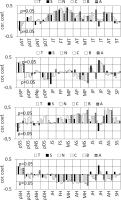
The results were similar in the case of rainfall of the previous September and of current May and July. The similar sensitivity of 6 pine populations to the amount of sunshine duration in April, May and August was also discovered. Similar patterns are connected with the effect of relative humidity of the previous September, and current February, March and April (Figs. 7 and 8).
The results of response function analysis performed for PC2 were also confirmed. It turned out that the incremental sensitivity of pine populations A and S to the temperature of the previous September and December and current June and July was different. It also differed from the sensitivity of the remaining pine populations (B, C, N and T) (Fig. 8). A difference was also observed regarding the effect of precipitation of January, April, May and June of the current year on increments of the A and S populations (Fig. 8). Differences were also found in the responses of pine trees on site A and S to the sunshine of the previous September and in the period between May and July of the year of ring formation. Also, the humidity of May, July and August had a different effect on the radial increment of pine trees on both of the above positions (Fig. 8). These climatic parameters were described by the other main component which differentiated the incremental rhythm of population A and C (Fig. 7).
The impact of rainfall, sunshine duration and relative humidity in the May of the ring formation year on pine radial increments was specific. On the one hand, the conditions above have had a similar effect on the incremental reactions of pines in all populations, as monthly values of these elements significantly correlate with PC1. On the other hand, they differentiate the incremental rhythm of each population, as they significantly correlate with PC2 (see Figs. 7 and 8). This paradox is explained by the results of the site chronology correlation analysis with the climatic parameters of May. It turned out that the direction of these relations is similar in 6 populations. However, increments of the S population of pines do not show a significant relationship with the above parameters of May, and population A has the strongest relation with the above (Fig. 8). It shows the similarity of the relation, but also highly diversified strength of these relations.
δ18O and δ13C chronologies
Analysis of stable isotope fractionation in annual tree rings of pines, growing in the sampling site where the strongest reduction of tree ring width was noted, is the complemental analysis to dendrochronological method. The pine tree-ring isotopic chronologies constructed in this study is a local grown pattern of the partial pine population (site C). These isotopic records (Fig. 8) are the result of the response of the trees to variations of various environmental factors such as climatic factors and human activities.
δ18O values varied between 27.4‰ to 31.8‰ (Fig. 9), whereas δ13C ranged from −24.6‰ to −22.9‰. The δ13C changes in the isotopic ratio of atmospheric CO2 due to global fossil fuels emissions ranged from 0.37‰ (in 1975) to 1.97‰ (in 2012). This correction lifts the δ13C values (Fig. 9). The δ13C values corrected for changes in the isotopic ratio of atmospheric CO2 ranged from −23.6‰ to −21.5‰.
Fig. 9
Stable carbon and oxygen isotopes chronologies. The raw δ13C values (dashed line) have been corrected (δ13Ccor values solid line) for changes in the isotopic ratio of atmospheric CO2.
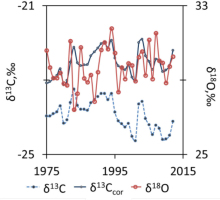
The analysis of combined measurement of carbon and oxygen isotopes ratio (Fig. 10) show positive significant correlation between δ13C and δ18O in the period of time from 1991–2012. No significant δ18O-δ13C relationship was observed between 1975 and 1990.
To describe the variation of the carbon and oxygen isotope composition of cellulose in annual tree-rings of pine caused by climate changes and human activities we used a model (Sensuła and Pazdur, 2013a, 2013b) based on multiple regressions (Fig. 11, Table 2):
where M is the month (from April to September), b is the regression coefficient for the following variables: T (mean of the monthly maximum temperatures), P (monthly precipitation sum), S (monthly hours of sunshine duration), H (mean of the monthly relative humidity), whereas ba corresponds to the interdependences between the monthly climate factors and other environmental changes.
Fig. 11
Measured (solid lines) and modelled (dashed line) values ot carbon and oxygen stable isotopic composition of α-cellulose samples extracted from annual tree rings of pine (1975–2012).
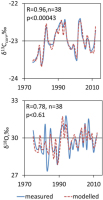
Table 2
The regression coefficient (Eq. 3.1) for the following variables: T (mean of the monthly maximum temperatures), P (monthly total precipitation), S (monthly hours of sunshine duration), H (mean of the monthly relative humidity). bi – multiple regression parameters. The test of significance gives a p-value (n=38).
The value of the correlation coefficient between the measured and modelled δ13C in α-cellulose is equal to 0.93, whereas the value of the correlation coefficient between the measured and modelled δ18O in α-cellulose is equal to 0.78.
Water use efficiency (WUE) and temperature anomaly
Diffuse air pollution (carbon dioxide) caused the variation in the ratio of water used in plant metabolism to water lost by the plant through transpiration (WUE). In the period of time from 1975 and 2012, according to NASA (2016) the global concentration of mid-tropospheric carbon dioxide ranged from ca. 330 ppm to ca. 393 ppm whereas the global surface temperature, relative to 1951–1980 average temperatures, ranged from −0.01°C to 0.76°C. During this period, the water use efficiency values increases from 94 to 122 µmol/mol (Fig. 12). It has been observed that with increase of CO2 emission also WUE increases. Water use efficiency might be strongly correlated with variability of the surface temperature (r=0.81)
Fig. 12
(a) Changes in water use efficiency (WUE) due to global distribution and variation of the concentration of mid-tropospheric carbon dioxide in parts per million (ppm) and (b) global surface temperature relative to 1951–1980 average temperature (c) correlation between water use efficiency and the variability of the global surface temperature.
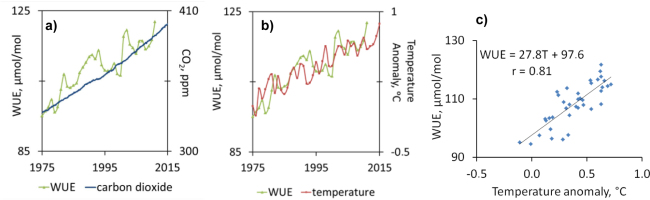
. Discussion
Tree-ring chronologies
Site chronologies of radial increment sizes represent incremental patterns of pines from partial populations. They illustrate the specific response of pine trees to various environmental factors, including the climate factor. It should be emphasized that in the years 1951–2012 the size of radial increments of the studied pine population changed. In the 1970s pines strongly reduced their radial increment. The reason for the reduction was a strong increase in industrial pollution. Despite this fact, pines in the area preserved their high year-to-year sensitivity to short-term impulses from the environment. It turned out, however, that the year-to-year rhythm of radial increments in particular populations was different. The reason was their different sensitivity to particular elements of the climate. In particular, two pine populations (S and A) were different in this respect – both, from each other and from the other four populations. They are located away from the main flow of air masses carrying pollutants from local factories. It is therefore assumed that the differences in the relations between climate and radial increments were the result of the varying pressure of pollution on the studied populations of trees. However, the influence of other factors, such as unrecognized differences in habitat conditions and the age of the trees cannot be excluded. Site A pines were in fact younger than others by approx. 20 years.
The differences in sensitivity of pines from A and S populations to the climatic elements described by PC2 were confirmed by the analysis of site chronology correlations. The Principal component analysis (PCA) is therefore an effective method of recognizing the similarities and differences in the sensitivity of trees to the climatic factor.
Interpretation of the modifying effect of pollutants on the relation between climate and tree increments is difficult. The influence of pollutants depends on the distance of trees from the emitters, the position of sites in relation to the direction of wind carrying pollutants, the age of trees and habitat conditions in which trees grow (Carrer and Urbinati, 2004; Yu et al., 2008; Friedrichs et al., 2009; Dauskane et al., 2011; Wilczyński, 2013; Sensuła et al., 2015a, 2015b).
A significant influence of the climatic conditions of the current and previous year on pine radial increments was observed. This is confirmed by numerous dendroclimatic studies on Scots pine (Vaganov, 1990; Lührte, 1991; Richter et al., 1991; Lindholm et al., 1996; Wilczyński and Skrzyszewski, 2003; Wilczyński, 2003, 2013; Pensa et al., 2005; Tuovinen, 2005; Juknys et al., 2014; Helama et al., 2013).
A strong positive correlation of site chronologies with the first main component indicates that pines showed similar sensitivity to climatic influences of PC1. Their role in shaping thickness increment of pines was independent of other elements of the environment. The results indicate that a cool, humid and rainy September meant that all the pine populations increased their increments in the following year. High temperatures and low air humidity in the autumn has a positive effect on the flowering of female flowers in the following year (Andersson, 1965; Fober, 1976). This has a negative impact on incremental growth of trees (Eis et al., 1965; Chałupka et al., 1976). On the other hand, cloudy, humid weather with plenty of rainfall has a beneficial effect on the number of buds of vegetative organs – needles and shoots (Hejnowicz, 1982). Therefore, the surface of assimilation and production of auxins increases, which speeds cambial divisions and growth of trees. It was also found that hot, short and sunny winters and dry air in this season positively influence the condition and incremental ability of 6 populations of Scots pine. Such conditions gave an earlier start to physiological processes that led to earlier and faster divisions of the cambium (Schober, 1951; Ermich, 1959; Wodzicki and Zajączkowski, 1983). A similar positive role was played by a dry, sunny and warm spring. These relations are confirmed in other dendroclimatic studies of the pine (Richter et al., 1991; Wilczyński and Skrzyszewski, 2003; Wilczyński, 2003; Juknys et al., 2014). The positive impact on the creation of the pine wood cells was also exerted by high rainfall in July of the year when the ring was formed, as they caused the extension of the period of intensive divisions of vascular cambium and the formation of large diameter cells. Low rainfall in summer is often a factor limiting the incremental growth of Scots pine basically throughout all of its range (Lührte, 1991; Lindholm et al., 1997; Irvine et al., 1998; Cinnirella et al., 2002; Wilczyński and Skrzyszewski, 2003; Pensa et al., 2005; Tuovinen, 2005; Pilcher and Oberhuber, 2007; Piovesan et al., 2008; Gruber et al., 2010; Juknys et al., 2014). Drought is a factor which often induces variability in growth patterns and mortality of Scots pine especially in dry areas (Bigler et al., 2006, Hereş et al., 2012, Herguido et al., 2016, Marqués et al., 2016). Heavy rainfall during the growing season and, consequently, increased air humidity enhances the flow of water into the cells increasing the pressure on the cell wall, thus stretching these and causing cambium cell growth and it also provides the substrates for the process of photosynthesis (Major and Johnsen, 2001). In turn, the deficit of water within this period reduces the turgor of the cell, decreasing the dividing activity of the vascular cambium (Pichler and Oberhuber, 2007). It also happens that excess of water harms trees, but mostly those on very humid sites (Cedro and Lamentowicz, 2011; Helama et al., 2013; Wilczyński, 2013).
δ18O and δ13C chronologies
The determination of properties of tree-rings is crucial for many applications in the investigation of local and global environmental changes. Since the beginning of the 20th century, there has been much discussion about how external environmental factors, including climate changes and anthropogenic effects affect the physiological processes that control tree growth (Schweingruber, 1996; DeVries et al., 2000; McCarroll and Loader, 2004; Pazdur et al., 2007, 2013; Sensuła et al., 2015a, 2015b; Sensuła, 2015). Through photosynthesis, plants convert CO2 and H2O to saccharides (C6H12O6), using light, and release oxygen to the atmosphere. The carbon of annual tree-ring has its origin in the CO2 of air, whereas the oxygen has its origin in the soil water and thus precipitation. The primary value of the isotopic records in tree-rings is not simply as samples of ancient air or water, but as sensitive bio-indicators of the way that the components of air and water have been changed by the trees in response to the environments in which they grown (Craig, 1954; Ehrelinger and Vogel, 1993; McCarroll and Loader, 2004; Savard, 2010; Sensuła, 2015).
The carbon isotopic composition (δ13C) of trees has been influenced by carbon isotopic composition of atmospheric CO2, diffusion of CO2 through stomata, and enzymatic discrimination during the irreversible step of CO2 fixation (Roden et al., 2000). The isotopic composition of plants varied due to emission of atmospheric pollution (Savard, 2010), such as for example sulphur dioxide (Rinne et al., 2010), carbon dioxide (Craig, 1954; McCarroll and Loader, 2004; Pazdur et al., 2007, 2013; Sensuła et al., 2011a, 2011b; Sensuła and Pazdur, 2013a, 2013b; Sensuła, 2015).
Trees grown at the higher level of CO2 concentration had a more negative δ13C than trees grown at the lower concentration. In pine, CO2 usually limits photosynthesis and, thus, an increase in CO2 results in greater photosynthetic rates. Raw δ13C data can be corrected to a preindustrial atmospheric δ13Ccor base value of 6.4% using the data from McCarroll and Loader (2004), due to decreasing δ13C in the air and the biosphere is associated with the increasing of anthropogenic CO2 in the atmosphere (Craig, 1954; Farquhar and Lloyd, 1993; Field et al., 1995; McCarroll and Loader, 2004; Pazdur et al., 2007, 2013; Keeling et al., 2010; Sensuła and Pazdur 2013a, 2013b; Battipaglia et al., 2014; Saurer et al., 2014). The analysis of the influence of pollution on stable isotopes composition will be a theme of the future study. Carbon dioxide (CO2) is an important heat-trapping (greenhouse) gas, which is released through human activities such as deforestation and burning fossil fuels, as well as natural processes such as respiration and volcanic eruptions. According to NASA (2016), and IPCC (2005), the extent of climate change effects on individual regions will vary over time and with the ability of different societal and environmental systems to mitigate or adapt to change. The IPCC predicts that increases in global mean temperature of less than 1 to 3 degrees Celsius above 1990 levels will produce beneficial impacts in some regions and harmful ones in others. Net annual costs will increase over time as global temperatures increase.
Researchers have used correlation analyses to determine which environmental parameters (precipitation, sunshine, humidity, and temperature) might be recorded in the isotopic composition of cellulose extracted from the annual growth rings (Schiegl, 1974; Gray and Thompson, 1976; Epstein and Yapp, 1977; Burk and Stuiver, 1981).
According to the scientific literature, the carbon isotopic composition in plant can vary with water stress and solar radiation and can be correlated with amount of rainfall, vapour pressure deficit, canopy position and hydraulic conductivity associated with tree height (Dongmann et al., 1974; Ehleringer, 1990; Ehrelinger and Vogel, 1993; Comstock and Ehleringer, 1992; Buchmann et al., 2002; Yoder et al., 1994; Barbour et al., 2002), whereas the oxygen isotopic composition of trees can be influenced by the oxygen isotopic composition of meteoric water and atmospheric vapour, atmospheric humidity and vapour pressure deficit (Farquhar and Lloyd, 1993; Roden et al., 2000). The oxygen isotopic composition of plant has been correlated with air temperature, relative humidity, transpiration rates and water balance, presumably all through contributions of source water (the xylem water in suberized stems) and leaf water enrichment (Ehrelinger and Vogel, 1993). In our studies, the impact of weather conditions on the isotopic concentration in pine was observed. The most significant climate factors (p<0.05, where p is the significance level) influencing δ13C is the monthly humidity in September (p=0.026), whereas the most significant climate factors influencing δ13Ccor are the monthly humidity in June (p=0.009) and September (p=0.001), sunshine in June (p=0.003), July (p=0.045) and September (p=0.013), whereas the most significant climate factors influencing δ18O in the investigated area is the mean of the May monthly maximum temperatures (p=0.043). The climatic signal record in trees stable isotopes chronologies may be masked by air contamination. Previous studies have shown weakened climate signal in δ13C and δ18O of α-cellulose of tree ring cellulose as well as deviant trends between δ13C and δ18O series caused by pollution during the 20th century (Rinne et al., 2010, Boettger et al., 2014, Sensuła, 2016b).
According to models (Farquhar and Lloyd, 1993; Scheidegger et al., 2000) a positive correlation of the δ18O and δ13C is predicted when Δ13C is driven by a strong stomatal reaction, whereas Amax (photosynthetic capacity) is relatively unaffected. Saurer et al. (1997) found a positive correlation between δ18O and δ13C of stem organic matter for pine trees growing in habitats with moist and dry soil conditions. The slopes in the δ18O-δ13C plots were found to be species-dependent and were interpreted as due to differing sensitivity to the moisture conditions. Generally, a positive correlation between oxygen and carbon isotopes might be expected in situations when water is not limiting, because there is no need to reduce water loss, which allows the stomata to operate over a wide range (Scheidegger et al., 2000).
A negative δ18O-δ13C relationship could be regarded as an indication that plants have stomata with a limited operational range (Scheidegger et al., 2000). According to Saurer and Siegwolf (2007) the stronger response of photosynthetic capacity (Amax) indicate that some species were able to enhance biomass accumulation due to increasing CO2 during the last decade, whereas other species responded more strongly with reduced stomatal conductance and less transpiration and water loss.
Spatial variability and temporal trends in water-use efficiency of European forests up until 2000AD has been studied in several tree species. Experimental results show that plants are able to increase their water-use efficiency (WUE) as CO2 levels rise (Ehlelinger et al., 1993; Morison, 1993; Field et al., 1995; Gagen et al., 2011; Saurer et al., 2014; Sensuła, 2015; 2016a). Increased atmospheric CO2 concentration may stimulate plant growth, indirectly through reduced plant water consumption and hence slower soil moisture depletion, and directly through enhanced photosynthesis (Morgan et al., 2004). According to NASA (2016) the variability in the global surface temperature might be due to increase of global CO2 emissions. According to NASA (2016) the land surface temperature is described as a measurement of how hot the land is to the touch. It differs from air temperature because land heats and cools more quickly than air. Most recent literature (for example, Stips et al., 2016) confirm that the total greenhouse gases (GHG), are the main drivers of the changing global surface air temperature. It has been observed that the elevated CO2 increases intrinsic water use efficiency (WUE) of forests, but the magnitude of this effect and its interaction with climate is still poorly understood. The analysis of the influence of variability of the global surface temperature due to increase of global CO2 anthropogenic emissions on WUE will be a subject of future analyses.
. Conclusions
The studied populations of Scots pine showed sensitivity to a wide range of meteorological factors occurring in a climate window covering the previous and current ring-forming year. Although pine is a boreal species, cold and long winters have a negative impact on its incremental activity. This relationship holds over the entire geographical range where pines can be found. Industrial pollution caused a reduction in the incremental growth of pines, but this fact does not have a significant impact on its short-term incremental sensitivity. Thanks to the above, a clear climate-radial increment relation was observed.
However, incremental rhythm of the studied pine populations was not identical. This was due to their different sensitivities to some factors of the climate. It was not possible, however, to unambiguously specify the factor that modified the relationship between climate and incremental growth in trees on particular sites. A different degree of the pollution pressure on trees might possibly be a factor shaping their different sensitivity to particular meteorological factors. These could also be other environmental factors. In order to obtain a reliable model based on the relationship between climate and incremental growth for this species, many different subpopulations should be studied on a given area. The principal component analysis proved to be an effective tool in identifying climate factors having a similar and different impact on the incremental rhythm of the studied pines.
The isotopic records in tree-rings can be a sensitive bio-indicators of the way that the components of air and water have been changed by the trees in response to the environments in which they grown. The carbon isotopic composition of trees has been influenced by carbon isotopic composition of atmospheric CO2. Also, the impact of weather conditions on the isotopic concentration in pine has been observed. The most significant climate factors influencing δ13Ccor are the monthly humidity and sunshine in summer. Whereas the most significant climate factors influencing δ18O in the investigated area was the mean of the May monthly maximum temperatures.
Based on measurements of δ18O and δ13C in two periods of time (1) prior to 1990s (where high pollution also recorded in tree ring width reduction) in and (2) after 1990s’ (when the pollution was reduced). Only in the period of time between 1990–2012, δ13C and δ18O showed a positive significant correlation that indicated a strong stomatal reaction, whereas Amax was relatively unaffected. It has been observed that water use efficiency might be strongly correlated with variability of the global surface temperature due to increase of global CO2 emission. The analysis of the influence of variability of the global surface temperature due to the increase of global CO2 anthropogenic emissions on WUE will be a subject of future study.